Solar Water Heating[edit | edit source]
The basic concept behind solar thermal water heating is the transfer of heat from solar radiation to a thermal fluid. In cases where the thermal fluid is not the domestic water source, heat is transferred from the thermal fluid to the domestic water source with a heat exchanger. Thermal storage is used to store the heat until it is supplied to the user. Sensing and pumping capabilities as well as the use of an auxiliary heat source improve system accuracy and ensure sufficient hot water delivery to the residence.
Solar water heating systems can be divided into passive and active systems, where passive systems do not require an external power source and active systems use electricity for pumping or sensing capabilities. Complex solar collectors improve system functionality and generally have low thermal losses at high temperatures.
Passive Systems[edit | edit source]
Passive solar water heating systems are appealing due to limited support requirements which reduce cost as well as maintenance requirements. The major limitation of is that they can typically only be installed in areas where there is no risk of freezing. Currently there are many regions where the likelihood of pipe freezing is unknown, thus passive systems cannot be installed. They are generally less efficient than active systems due to their lack of sensing and pumping capabilities, and may not meet the heat demand of a residence if solar resource is insufficient.
Integral Storage Collector Systems (ICS)[edit | edit source]
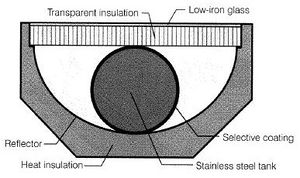
]
Integral storage collector systems are the most basic configuration of a passive heating system, and function by combining the hot water storage tank with the collector. These systems generally cost less than other passive water heating systems due to increased simplicity and limited component requirements.
The basic ICS system consists of a hot water tank with an insulated back side, where reflectors inside the tank serve as absorbers and reflect light to the stored water. The tank is covered with a transparent insulating cover to minimize evaporation and prevent water contamination. Figure 3 shows a side view of an ICS system.
The use of a transparent cover for an integral storage collector system introduces the potential for large heat losses. Recent technologies in transparent insulation materials have introduced low-transmittance solutions to minimize losses at the expense of cost increases. The use of a vacuum inside the tank can minimize convective losses, again at the expense of system complexity and cost.
The opportunity for freeze protection is limited for ICS systems due to the basic configuration of these systems. Basic freeze protection can be provided by rooftop mounting, double or triple glazing on the transparent covering, freeze tolerant tank construction or the implementation of an auxiliary heat source. Generally speaking, however, the use of integral storage collector systems is limited to mild climates unless being used as a pre-heat for conventional hot water systems.
Thermosyphon Systems[edit | edit source]

]
Thermosyphon water heating systems function by exploiting water density changes to circulate heated water from a collector to a thermal storage unit, as shown in Figure 4. The collector is installed below the storage tank, which draws water from the bottom of the tank to the collector where it is heated. The heated water then rises back to the storage tank due to its lower density. This cycle continues until the water temperature is constant throughout the tank.
The thermal reservoir for a thermosyphon must be separated from the collector and located a minimum of 18" above the collector for adequate circulation [21]. In general, best practice entails a larger height difference between collector and storage tank to ensure the system doesn't run backwards at night. This is often achieved by installing the storage tank on the roof, which brings about further structural design considerations.
The major disadvantage of a thermosyphon system is its lack of reliable freeze protection. These systems are inert and cannot react quickly to changes in solar radiation. Collector efficiency generally decreases with high temperatures in the solar cycle, thus these systems may not meet hot water demands for a particular application.
One way to increase the versatility of a thermosyphon heating system is to install a solenoid valve, syphon breaker or air vent to completely drain the collector in the event of freezing weather. These additions increase the complexity of the system and require some, however they offer some degree of freeze protection in regions where a passive system may be otherwise impossible.
Another method for freeze protection in thermosyphon systems is through the use of a thermal fluid (water with antifreeze, for example) in conjunction with a heat exchanger. In colder regions, the thermal fluid may circulate through a tank jacket to provide additional freeze protection. For these systems, piping exposed to the environment must be protected from freezing by use of electric heat tape or automatic drip valves.
The basic thermosyphon system is most applicable for mild climates where freezing temperatures are unlikely. For this reason, these systems are particularly popular in Australia, Japan and Israel. Until a more reliable form of freeze protection is introduced, the widespread use of thermosyphon domestic water heating systems is unlikely in temperate and cold regions.
Phase Change Systems[edit | edit source]

]
Phase change solar water heating systems operate in a similar fashion to thermosyphon systems, however the thermal fluid is allowed to change phases inside the system. The thermal fluid is heated up in the collector and allowed to vaporize. It then travels upwards to the thermal storage, where it condenses and transferred stored heat to the domestic water supply. The thermal fluid then flows back into the collector by gravity. This process can be observed in Figure 5.
Generally, it is useful to install temperature sensors in phase change heating systems to ensure refrigerant vapor temperature is higher than hot water temperature in the tank. When hot water temperature exceeds that of the thermal fluid vapor, the cycle must be stopped to prevent the system from running backwards.
As with thermosyphon systems, the thermal storage tank in a phase change heating system must be located above the collector to ensure proper functionality. Increased temperature accuracy requirements of a phase change system generally require some sensing capabilities, and the use of a pump can further increase functionality.
Active Systems[edit | edit source]
Active solar water heating systems are more common than passive systems due to their increased installation and application versatility. They offer improved circulation, increased sensing and controlling capabilities, as well as improved and more reliable methods of freeze protection.
Forced Circulation Systems[edit | edit source]
Forced circulation systems use a pump to move heated water through the system, eliminating the need for a height difference between the collector and the thermal reservoir. The use of a pump allows flow rate adaptation to available solar radiation, and thus can ensure adequate water heating. In general, lower flow rates compromise pump efficiency and high flow rates have higher power requirements; flow rate should be monitored to balance these considerations. Temperature sensing can be employed to monitor temperatures in the collector and storage tank, and can dictate whether or not the pump should run based on temperature difference.
Direct Heating Systems[edit | edit source]

]
Direct solar water heating refers to direct heating of the domestic water source by the sun. In general, these systems offer limited methods of freeze protection.
A single tank direct heating system consists of a single tank where potable water is circulated through the collector to a single storage tank. An auxiliary heat source is generally installed at the top of the tank to ensure tank stratification for proper system functionality. Cold water is supplied to the collector from the bottom of the tank, and the hot water leaving the collector enters the tank at the top. Sensing and controller capabilities allow controlled circulation when tank stratification is insufficient for adequate water heating. This configuration can be found in Figure 6.
Freeze protection for direct heating systems is limited to recirculation and draindown systems. Recirculation can be used in regions where freezing temperatures are less of a concern. These systems function by continually circulating water from the storage tank through the collector to prevent system freezing. Temperature sensors and controls can prompt recirculation in cooler weather.
Draindown systems contain motorized or solenoid valves which are used to drain the system when freezing is a possibility. In freezing conditions, the water supply to the collector is cut off and a drain valve is opened; air is allowed to enter the collector and associating piping to avoid damage due to freezing.
Indirect Heating Systems[edit | edit source]

]
Indirect water heating methods were developed to provide a more reliable method of freeze protection than what is offered by direct solar water heating systems. The general design consists of a non-freezing thermal fluid which is heated in the collector, and heat is transferred from the thermal fluid to the domestic hot water source in a heat exchanger as found in Figure 7. These systems may contain recirculation or draindown systems as described above to further protect against freezing.
Important considerations for indirect water heating systems include increased pumping requirements, heat transfer characteristics, capital costs and ongoing maintenance requirements. The expansion of the heat transfer fluid must be taken into account when designing an indirect heating system, as well as location and installation of check valves. It is imperative that the thermal transfer fluid is in a closed loop, as the pressure of city water supply will otherwise prevent draining in the event of a freeze.
It is possible to use air as the thermal fluid, however efficiency can be limited due to its lower heat capacity. Additional considerations such as the cost of blower power, heat exchanger efficiency and system air tightness must be compared to the costs of using a traditional liquid thermal transfer fluid.
Design Considerations[edit | edit source]
Solar water heating systems must be designed primarily as a function of available solar irradiation and hot water demand. Ultimately, the implementation of a solar water heating system depends on its ability to save the user money, protect the environment and limit use of fossil fuels. Once these design parameters are well understood, an appropriate solar water heating system may be selected.
Proper design selection is essential to ensure maximum gain to the user. Solar domestic water heating systems are only cost-effective when properly designed, as performance depends directly on installation configuration. The selected design can be validated by knowing available solar insolation, heat and load demands and solar fraction. Correct design installation is essential to proper functionality of any system.
Table 1 below outlines some basic considerations which should be taken into account when selecting a solar water heating system for a particular application.

]
Other operating considerations include freeze protection, a means for rejecting excess heat to prevent system boilover, the potential need for auxiliary heat back-up if the solar system fails and contamination prevention for indirect heating with a non-potable thermal fluid. These considerations are explored in more detail below.
Solar Resource[edit | edit source]
Other operating considerations include freeze protection, a means for rejecting excess heat to prevent system boilover, the potential need for auxiliary heat back-up if the solar system fails and contamination prevention for indirect heating with a non-potable thermal fluid. These considerations are explored in more detail below.
Heat and Load Demand[edit | edit source]

]
Hot water draw can vary greatly from site to site and season to season, and also based on the application (residential vs. commercial, steam vs. hot water). While hot water use can be estimated based on usage at other locations, the only way to properly quantify hot water demand for a specific site is by measuring use over time. It is generally accepted that the highest hot water usage is between 6am and 10am, with a second smaller peak from 2pm to 10pm, as found in Figure 8.
As a point of reference, Table 2 below outlines the hot water demand of a residential building in Germany in 1982.

]
While these values can be used as a template for system design, it is important to note that unexpected load variations from day to day can significantly affect system performance. Other demand considerations include heat losses, heat-up considerations and solar collector power output effect on auxiliary heat needs.
Once the hot water demands of a particular location are well-defined, the associated heat demand can be calculated as follows:

This value can be used to determine the solar capabilities of a particular region with respect to demand for a specific application.
Hot Water Demand in the United States[edit | edit source]
It is estimated that a typical American family of 4 uses 50-100 gallons of hot water per day, equating to approximately 50,000 Btu/day [21]. In sunny regions, one square foot of collector area can typically harness 500 Btu/day, making a solar water heating system applicable for these regions and minimizing the need for auxiliary energy.
A solar water heating system was installed in Salt Lake City, Utah for a residence of 12 young girls and their children [4]. Measured hot water demand was as follows:

]
Again, these values can be used as guidelines, however accurate hot water demand for a specific location can only be verified by direct measurement.
Hot Water Demand in South Africa [25][edit | edit source]
One appealing aspect of solar water heating systems is their potential application in developing regions. It has been suggested that Americans use up to seven times the amount of hot water that is used in other developed European countries, thus hot water use cannot be extrapolated to estimate use in developing area. Cost is of particular importance in these environments, as installation will only be considered if significant cost savings are achievable.
A study was performed by Joshua Meyer for the ASHRAE (American Society of Heating, Refrigerating, and Air-Conditioning Engineers) on hot water demand in South Africa as a function of living space. South Africa has a mild climate, thus water heating in middle to upper-class homes is the largest source of energy consumption in the domestic sector, accounting for 40-50% of total energy costs for a household.
Current methods for water heating in South Africa include electric element heating, gas heating, heat pumps and some solar heating, where electricity dominates as the main method for domestic water heating. There are a limited number of homes equipped with solar water heating systems due to their high capital cost and the low cost of electricity.
In 1996, hot water consumption over a year was measured by the Johannesburg Metropolitan Area for 770 homes, ranging from affluent residences with readily available hot water to shacks outside the city center where water is heated over an open fire. It was found that daily hot water consumption was higher in higher income homes, and that hot water consumption increases approximately 70% in winter months, especially by upper-class (low occupant density) homes. In general, hot water use spikes occur from 7am to 9am, and again from 9pm to 11pm.
Daily consumption was measured based on residential occupant density, where lower-income homes generally have higher occupant density. Table 4 below lists estimated daily residential consumption rates by occupant density.
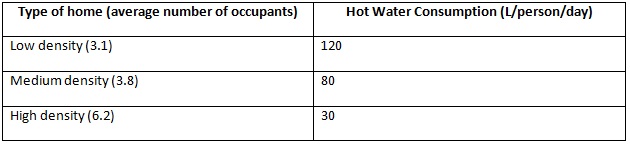
]
While the values observed in this study cannot be applied directly to the design of a solar water heating system in all developing areas, they are qualitatively useful at the beginning of the sizing and design process.
Solar Fraction and Cycle Efficiency[edit | edit source]
Solar fraction refers to the percentage of overall heat demand that is provided by the solar system, defined mathematically as:

A solar fraction of 50-60% is desirable for domestic water heating applications, as this allows a compromise between heating capability and cost considerations [28]. In regions of high solar irradiation, the solar fraction may be much higher, especially in areas where there is minimal temperature variation from season to season.
After construction considerations, solar fraction is the dominant factor on solar cycle efficiency, where overall system efficiency increases with increasing solar fraction. Solar cycle efficiency refers to total efficiency for the solar thermal system, defined as mathematically as:
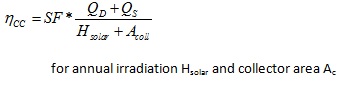
Temperate regions generally have a solar cycle efficiency of 20-50% due to a lower available solar fraction [28].
Design Validation Techniques[edit | edit source]
Validation of a selected solar water heating system can be performed to ensure adequate system performance for a specific application. There are two basic methods for optimization: correlation-based methods which base functionality on utilizability, and simulation-based methods using computer programs. Correlation-based methods are limited because of the complexity of solar water heating systems; most methods only allow identification of a single system design by optimizing one function. Computer programs offer a more comprehensive approach for design validation, however their use may increase cost of a particular system.
While there is no widely-accepted method for design validation, a few techniques have been proposed in industry and in the scholarly arena.
Model for Determination of Design Space [19][edit | edit source]
One technique proposed by Kulkarni, Kedare and Bandyopadhyaya incorporates design constraints to compare solar water heating designs based on balancing collector area and storage volume. It is found that there is a minimum and maximum possible storage volume for a given solar fraction and collector area. Similarly there exists a minimum and maximum possible collector area for a given solar fraction and storage volume. The overall system is then optimized by minimizing annual costs.
Minimum collector area is determined by finding the smallest possible storage volume to still meet domestic water heating demands. A collector with smaller area than this specified minimum will not meet the thermal requirements of the water heating system.
Larger tank volumes have fewer temperature fluctuations, thus lower temperature water is sent to the collector which increases cycle efficiency. Conversely, increased surface area of these units increases thermal losses which decreases thermal storage efficiency. As storage volume increases, increases in collector efficiency before less noteworthy and changes in storage efficiency become more considerable. Minimum storage volume is defined by the smallest acceptable storage volume to prevent system boiling.
These qualitative considerations can be combined to determine acceptable design limits. Stored water must meet load requirements without boiling; any design which fits between these temperature constraints represents a design which satisfies load requirements and temperature constraints.
While the basic methodology proposed in this paper considers only temperature and load constraints, the model can be extended to include cost considerations, solar fraction and year-to-year performance data, which can be useful for economic optimization of a specific design. Incorporation of additional constraints will increase the number of required iterations, however the result is a collection of acceptable designs for one particular application.
Results using this methodology cannot be extrapolated globally due to uncertainty in solar insolation data, system parameters and cost data, however it still provides a useful way to determine a collection of acceptable designs for a particular domestic water heating application. This technique is especially useful in retrofit applications by incorporating existing constraints into the global model. Uncertainties associated with system parameters may cause variance between a globally optimized system and actual field results, thus results are more effectively applied qualitatively.
Procedure for Life Cycle Assessment [3][edit | edit source]
The completion of a life cycle assessment is useful to understand the economics of solar water heating and as to identify major contributors to environmental impacts and compare design options for a particular application. A complete life cycle assessment requires in depth planning, inventory analyses, impact assessments and improvement analyses, however a more generalized approach is used in "Life Cycle Assessment of Built-in-storage Solar Water heaters in Pakistan" (Asif and Muneer).
The purpose of this study was to determine the effect of including fins on a collector for a conventional integrated collector storage system. The comparison process was simplified by installing two identical systems were constructed: one using the traditional collector design and one with fins. Both systems were fully instrumented to measure hourly variation of ambient air and water temperatures in addition to tank stratification temperatures. Data was collected for four summer months and output power for each system was compared.
Table 5 below outlines system characteristics of each design.
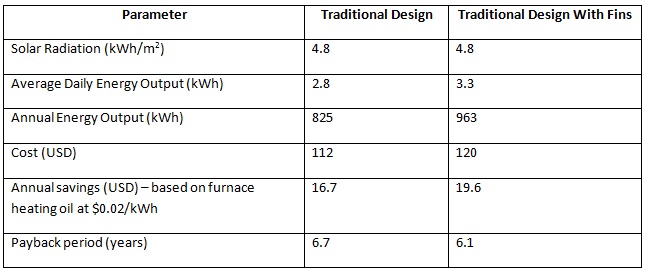
]
The parameters above describe the more common measurement tools used to perform a life cycle assessment of a particular system. It is also beneficial to consider the energy demand and savings achieved by installing a solar water heating system.
Based on the materials used for the solar water heaters (stainless steel, glass, rubber, timber and glass wool insulation), energy values per mass were determined as found in Table 6 below. This information combined with average amounts of transmitted energy can be used to estimate the energy payback period for both systems.

]
The methodology used to determine energy demand and savings for these two systems can be applied to examine carbon emission savings. It is estimated that the use of solar water heating systems reduces carbon emissions on the scale of 0.02kg/MJ. This estimation is extrapolated for both designs as found in Table 7 below.

]
The results found in this paper cannot be applied to all solar water heating systems, however the methodology can be extended to compare an iterated system to a more basic or traditional system.
Installation Considerations[edit | edit source]
Correct installation of a solar water heating system is required for proper system functionality. Table 8 below describes some specifics important points for system installation.
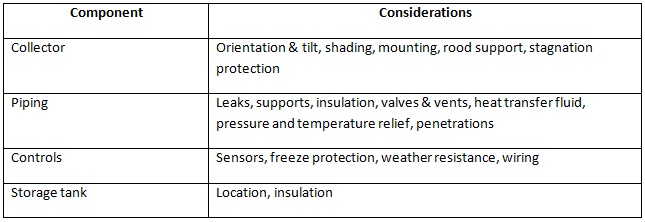
] These considerations can be further divided as found below.
Freezing[edit | edit source]

]
One important design consideration is the potential for freezing in temperate or cold climates. Exposed piping is at the greatest risk for freezing and permanent damage can be caused to the collector during freezing weather. For this reason, the installation of passive systems is limited to regions where freezing weather is not a concern.
Daytime water draw has a large effect on the likelihood of system freezing and thus must be considered when designing the freeze-proofing characteristics of a system. Increased hot water draw can replace cold water on the verge of freezing with warmer water, which also may melt existing ice buildup. Other factors which may inhibit pipe freeze include conduction, single-pipe convection and overall solar gain.
A variety of studies have been performed to investigate the mechanism and likelihood of pipe freezing. A University of Illinois study by Gordon in 1996 revealed that pipe burst due to freezing is only caused when two complete ice blockages occur. Another study at the University of Wisconsin by Beckman and Bradley in 2000 created a time-to-freeze map of the United States for a wide variety of pipe configurations. Salasovich, Burch and Barker of the National Renewable Energy Laboratory used the information from these studies to create a more descriptive contour map which details the likelihood of pipe freezing based on real weather data, hot water draw information, vacation periods with no hot water draw and pipe geometry and insulation, found in Figure 9.
While the figure above provides useful estimations of where pipe freezing is a concern, it can only be used qualitatively. Additionally, the figure above is only applicable for metal pipes; polymer pipes have higher yield strain and will not necessarily burst due to complete blockage.
Sensor Installation[edit | edit source]
Sensors and controls can be installed on a solar water heating system to more accurately monitor and adjust heating characteristics based on feedback. It is especially important to ensure proper sensor selection and installation, well as to determine optimum sensor location. Best practice entails that the estimated temperature difference should not exceed the precision range of the sensor, and the device should not be in direct contact with the fluid being measured.
Collector Array Performance[edit | edit source]
When possible, it is preferable to have solar collectors face due south and have a tilt angle approximately equal to the installation latitude [21]. Additional architectural considerations may be required in locations which prevent these installation guidelines, such as tall buildings or areas with excessive shade.
Improper piping of collector arrays can cause flow imbalance which will eventually overheat sections of the collector and decrease overall collector efficiency. This can be avoided by limiting total piping length via series installation of the collectors. This may solve some efficiency issues, however collectors should not be installed with more than three in series due to increased pressure requirements and excessively high inlet temperatures for the final collectors.
A final design consideration for large collector arrays is the increased difficulty to monitor and detect circulation issues. More recent infrared thermographic technologies can be used to show temperature distribution throughout the system which can indicate flow imbalances, air blockages or broken collectors.